4.3 Geological Renaissance of the Mid-20th Century
Two key areas of research ultimately led to the acceptance of continental drift, and the formulation of plate tectonic theory. One was the study of paleomagnetism, the record of Earth’s magnetic field through time. The other was exploration of the ocean floor.
Paleomagnetism (Remnant Magnetism)
![Figure 4.6 Rock layers recording remnant magnetism. The red arrows represent the direction of the vertical component of Earth's magnetic field. The oldest rock has a magnetic dip characteristic of the southern hemisphere, but over time the dip changes, indicating that the rocks moved toward magnetic north. [SE]](https://opentextbc.ca/kzlab/wp-content/uploads/sites/360/2018/08/image0151.png)
When rocks form, some of the minerals that make them up can become aligned with the Earth’s magnetic field, just like a compass needle pointing to north. This happens to the mineral magnetite (Fe3O4) when it crystallizes from magma. Once the rock cools the crystals are locked in place. This means that if the rock moves, the crystals can’t realign themselves, and they retain a remnant magnetism. This would be like jamming your compass needle so that if you turned away from north, the needle would turn with you rather than continuing to point north.
Rocks like basalt, which cool from a high temperature and commonly have relatively high levels of magnetite, are particularly susceptible to being magnetized in this way. However, even sediments and sedimentary rocks can take on remnant magnetism as long as they have small amounts of magnetic minerals, because the magnetic grains can gradually become lined up with Earth’s magnetic field as the sediments are deposited.
By studying both the horizontal and vertical components of the remnant magnetism, one can tell not only the direction to magnetic north at the time of the rock’s formation, but also the latitude where the rock formed relative to magnetic north. Remember that the vertical component of the magnetic field points more sharply downward the closer it is to the magnetic north pole. Figure 4.9 shows the vertical component of remnant magnetism in a sequence of rocks. Notice that the arrow starts out at 500 Ma pointing slightly upward. This means that the rocks were in the southern hemisphere. As the rocks get younger, the arrow tilts toward horizontal, and then points downward. This indicates that the rocks were getting progressively closer to the north magnetic pole.
Apparent Polar Wandering Paths
In the early 1950s, a group of geologists from Cambridge University, including Keith Runcorn, Ted Irving,[1] and several others, started looking at the remnant magnetism of Phanerozoic British and European volcanic rocks, and collecting paleomagnetic data. Using an analysis similar to that in Figure 4.9, they noticed that rocks of different ages sampled from the same general area showed very different magnetic pole positions (the green line in Figure 4.10). They assumed this meant that Earth’s magnetic pole had moved around significantly over time along polar wandering paths, rather than staying close to the geographic north pole as it does today. At the time, geophysical models suggested that the magnetic poles did not need to be aligned with the rotational poles, so this wasn’t an unreasonable conclusion, given what was known.
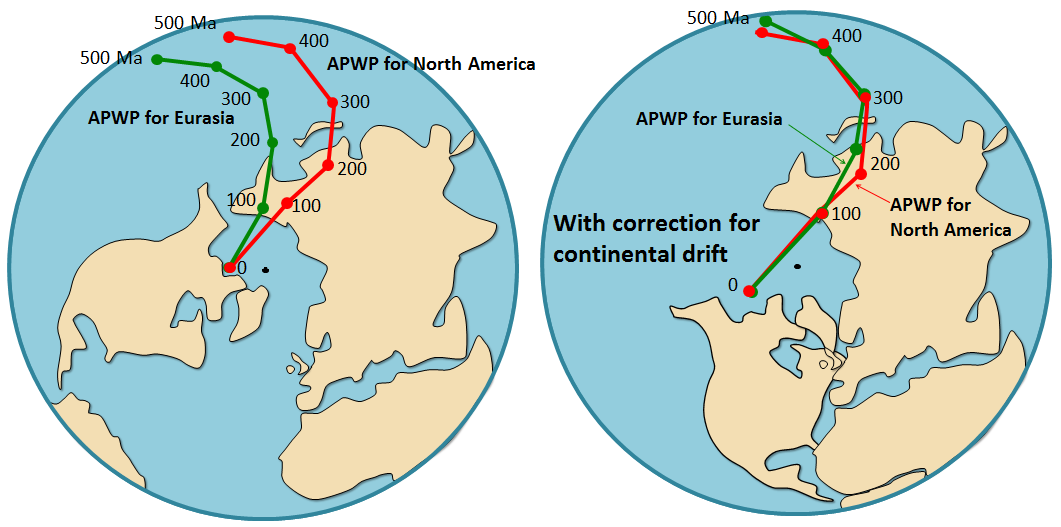
Runcorn and colleagues extended their paleomagnetic studies to North America, and began to realize that their initial conclusion had a problem. Notice that on the right of Figure 4.10 the polar wandering path for North America (in red) does not match the path for Eurasia (in green). For example, data from North America suggest that 200 Ma ago, magnetic north was somewhere in China, whereas data from Europe said it was in the Pacific Ocean. There could only have been one magnetic north pole position at 200 Ma, therefore the only way to explain the discrepancy was if Europe and North America moved along different paths during this time while the pole stayed in more or less the same location.
The polar wandering paths were not actually records of the pole moving, they just looked that way, so the paths are now referred to as apparent polar wandering paths (APWP). Subsequent paleomagnetic work showed that unique apparent polar wandering paths can be derived from rocks in South America, Africa, India, and Australia. In 1956, Runcorn became a proponent of continental drift. There was simply no other way to explain the data.
This paleomagnetic work of the 1950s was the first new evidence in favour of continental drift, and it led a number of geologists to start thinking that the idea might have some merit. Nevertheless, for a majority of geologists, this type of evidence was not sufficiently convincing to get them to change their views.
Concept Check: Clues from Paleomagnetism on Wandering Continents
Write the words into the correct boxes to complete this summary.A history of Earth’s ancient magnetic field, determined through the study of , is preserved in rocks as . Magnetic minerals align with , and once a rock solidifies, the minerals are locked in place. This is like gluing a compass needle down. When the minerals (or your modified compass) are moved, they no longer respond to Earth’s magnetic field.
A key discovery was that magnetic minerals on different continents show different paths. This doesn’t make sense, because there can only be one north pole at a time. The only explanation is that the were moving. This is why these paths are now called paths.
Fill-in-the-blank options:
- continents
- remnant magnetism
- paleomagnetism
- polar wandering
- magnetic north
- apparent polar wondering
To check your answers, navigate to the below link to view the interactive version of this activity.
Ocean Basin Geology and Geography
During the 20th century, our knowledge and understanding of the ocean basins and their geology increased dramatically. Before 1900 we knew virtually nothing about the bathymetry (the hills and valleys of the ocean floor) and geology of the oceans. By the end of the 1960s, we had detailed maps of the topography of the ocean floors, a clear picture of the geology of ocean floor sediments and the solid rocks beneath them, and almost as much information about the geophysical nature of ocean rocks as of continental rocks.
Acoustic Depth Sounding
Up until the 1920s, ocean depths were measured using weighted lines dropped overboard. In deep water this is a painfully slow process and the number of soundings in the deep oceans was probably fewer than 1,000. That is roughly one depth sounding for every 350,000 square kilometres of the ocean. To put that in perspective, it would be like trying to describe the topography of British Columbia with elevation data for only a half a dozen points!
The voyage of the Challenger in 1872 and the laying of trans-Atlantic cables had shown that there were mountains beneath the seas, but most geologists and oceanographers still believed that the oceans were essentially vast basins with flat bottoms, filled with thousands of metres of sediments.
Following development of acoustic depth sounders (Figure 4.11) in the 1920s, the number of depth readings increased by many orders of magnitude, and by the 1930s there was no doubt that major mountain chains ran through all of the world’s oceans. During and after World War II, there was a well-organized campaign to study the oceans, and by 1959, sufficient bathymetric data had been collected to produce detailed maps of all the oceans (Figure 4.12).
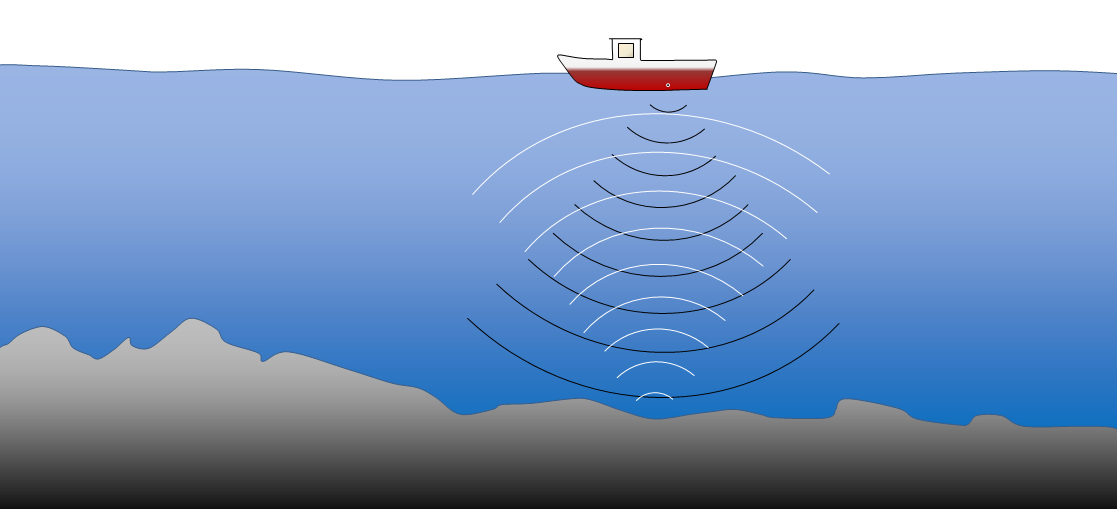
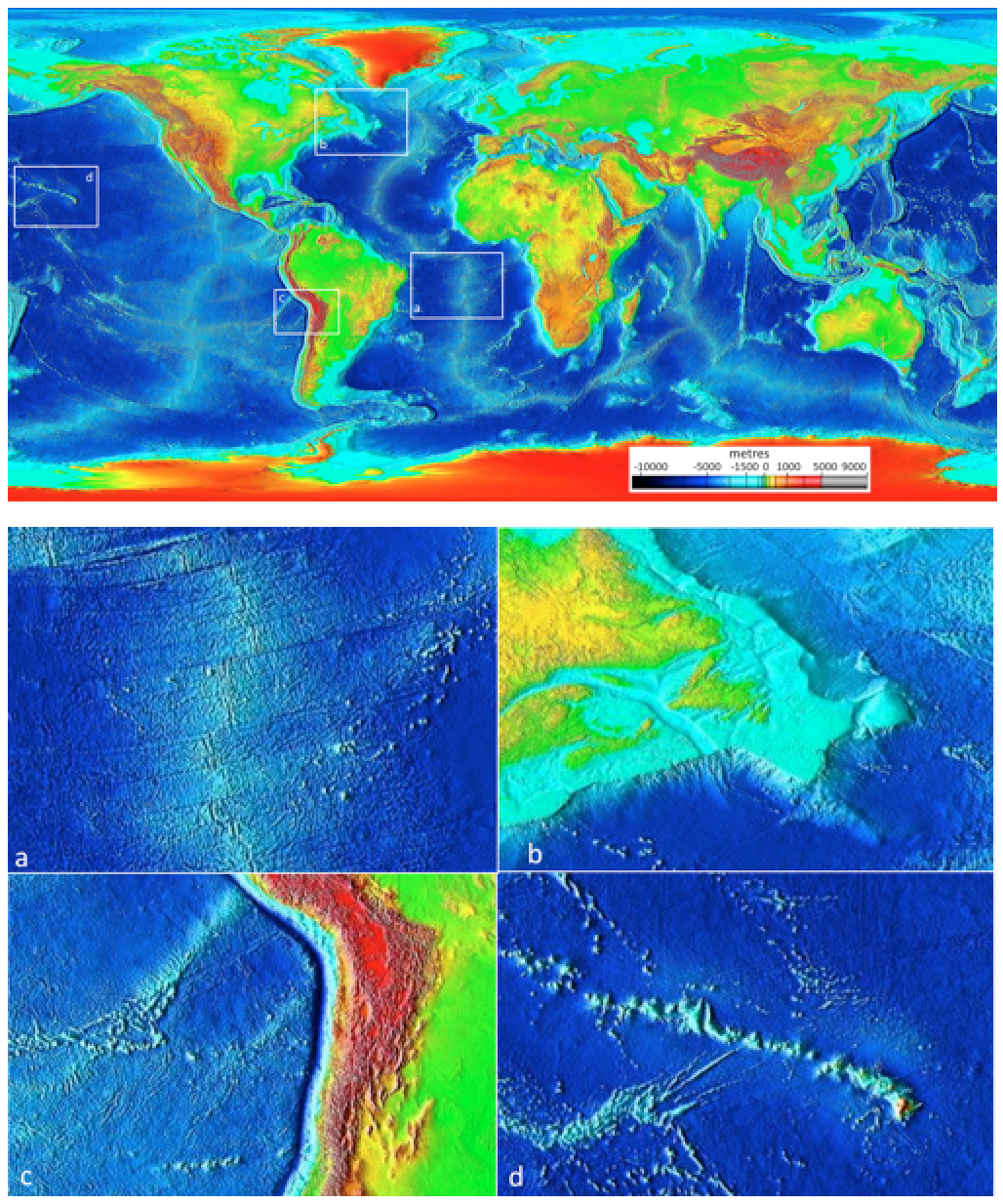
The important physical features of the ocean floor are:
- Extensive linear ridges (commonly in the central parts of the oceans) at depths of 2,000 to 3,000 m (Figure 4.12, a)
- Fracture zones perpendicular to the ridges (Figure 4.12, a)
- Deep-ocean plains at depths of 4,000 to 5,000 m (Figure 4.12, a and d)
- Relatively flat and shallow continental shelves with depths under 500 m (Figure 4.12, b)
- Deep trenches (up to 11,000 m deep), most near the continents (Figure 4.12, c)
- Seamounts and chains of seamounts (Figure 4.12, d)
Seismic Reflection Sounding
Seismic reflection sounding involves transmitting high-energy sound bursts and then measuring the echoes with a series of receivers called geophones towed behind a ship. The technique is related to acoustic sounding as described above, however, much more energy is transmitted and the sophistication of the data processing is much greater. As the technique evolved, and the amount of energy was increased, it became possible to see through the sea-floor sediments and map the bedrock topography and crustal thickness. This allowed sediment thicknesses to be mapped (Figure 4.13).
![Figure 4.10 Map of global sediment thickness. [Source: NOAA, http://1.usa.gov/1Ywxxz6]](https://opentextbc.ca/physicalgeologyh5p/wp-content/uploads/sites/360/2021/08/sedthick9.jpg)
It was soon discovered that although the sediments were up to several 1000s of m thick near the continents, they were relatively thin — or even non-existent — along ocean ridges (Figure 4.14). The seismic studies also showed that the crust is relatively thin under the oceans (5 km to 6 km) compared to the continents (30 km to 60 km) and geologically very consistent, composed almost entirely of basalt.

Heat Flow Rates
In the early 1950s, Edward Bullard—who spent time at the University of Toronto but is mostly associated with Cambridge University—developed a probe for measuring the flow of heat from the ocean floor. Bullard and colleagues found higher than average heat-flow rates along the ridges, and lower than average rates in trenches. These data were interpreted as evidence of mantle convection, with areas of high heat flow corresponding to upward convection of hot mantle material, and areas of low heat flow corresponding to downward convection.
Earthquake Belts
With developments of networks of seismographic stations in the 1950s, it became possible to plot the locations and depths of both major and minor earthquakes with great accuracy. A remarkable correspondence was observed between earthquake locations and both the mid-ocean ridges and the deep ocean trenches. In 1954 Gutenberg and Richter showed that the ocean-ridge earthquakes were all relatively shallow, and confirmed what had first been shown by Benioff in the 1930s, that earthquakes in the vicinity of ocean trenches were both shallow and deep, but that the deeper ones were situated progressively farther inland from the trenches (Figure 4.15).
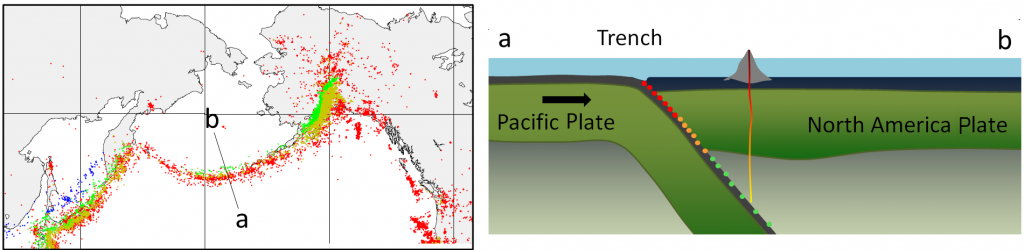
Magnetic Stripes on the Sea Floor
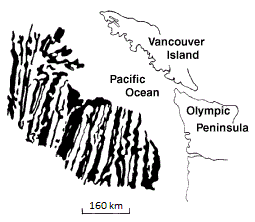
In the 1950s, scientists from the Scripps Oceanographic Institute in California persuaded the United States Coast Guard to include magnetometer readings on one of their expeditions to study ocean floor topography. The first comprehensive magnetic data set was compiled in 1958 for an area off the coasts of BC and Washington State. This survey revealed a bewildering pattern of low and high magnetic field intensity in sea-floor rocks (Figure 4.16). When the data were first plotted on a map in 1961, nobody understood them — not even the scientists who collected them. Many 1000s of km of magnetic surveys were conducted over the next several years.
The wealth of new data from the oceans began to significantly influence geological thinking in the 1960s. In 1960, Harold Hess from Princeton University, advanced a hypothesis with many of the elements that we now accept as plate tectonics. He maintained some uncertainty about his proposal however, and in order to deflect criticism from mainstream geologists, he labelled it “geopoetry.” In fact, until 1962, Hess didn’t even put his ideas in writing—except internally to the U.S. Navy (which funded his research)—but presented them mostly in lectures and seminars.
Hess proposed that new sea floor was generated from mantle material at the ocean ridges, and that old sea floor was dragged down at the ocean trenches and re-incorporated into the mantle. He suggested that the process was driven by mantle convection currents, rising at the ridges and descending at the trenches (Figure 4.17). He also suggested that the less-dense continental crust did not descend with oceanic crust into trenches, but that colliding landmasses were thrust up to form mountains.
Hess’s hypotheses formed the basis for our ideas on sea-floor spreading and continental drift, but did not go so far as to claim that the crust is made up of separate plates. The Hess model was not roundly criticized, but also not widely accepted, partly because evidence was still lacking.
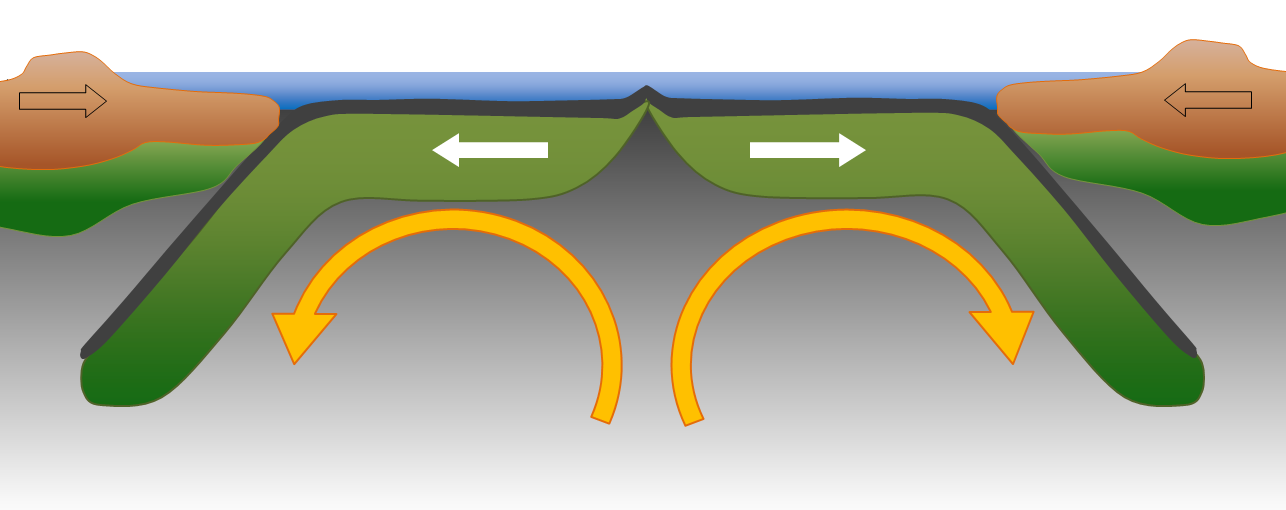
Collection of magnetic data from the oceans continued in the early 1960s, but the striped patterns remained unexplained. Some assumed that, as with continental crust, the stripes were related to compositional variations in rock, such as variations in the amount of magnetite. The first real understanding of the significance of the striped anomalies was the interpretation by Fred Vine, a Cambridge graduate student. Vine was examining magnetic data from the Indian Ocean and, like others before him, noted that the magnetic patterns were symmetrical on either side of the ridge.
At the same time, other researchers led by groups in California and New Zealand were studying the phenomenon of reversals in Earth’s magnetic field. They were trying to determine when such reversals had taken place over the past several million years by analyzing the magnetic characteristics of hundreds of samples from basaltic flows. As discussed in Chapter 3, Earth’s magnetic field periodically weakens, then becomes virtually non-existent before becoming re-established with the reverse polarity. During periods of reversed polarity, a compass would point south instead of north.
The time scale of magnetic reversals is irregular. The present “normal” event, known as the Bruhnes magnetic chron, has persisted for about 780,000 years. It was preceded by a 190,000-year reversed event; a 50,000-year normal event known as Jaramillo; and then a 700,000-year reversed event (see Figure 3.16).
In a paper published in September 1963, Vine and his PhD supervisor Drummond Matthews proposed that the patterns associated with ridges were related to the magnetic reversals, and that oceanic crust created from cooling basalt during a normal event would have polarity aligned with the present magnetic field, and would produce a positive anomaly (a black stripe on the sea-floor magnetic map). Oceanic crust created during a reversed event would have polarity opposite Earth’s present field and thus produce a negative magnetic anomaly (a white stripe). The same idea had been put forward a few months earlier by Lawrence Morley, of the Geological Survey of Canada. However, Morley’s papers submitted earlier in 1963 to Nature and The Journal of Geophysical Research were rejected. The idea is sometimes referred to as the Vine-Matthews-Morley (VMM) hypothesis.
Vine, Matthews, and Morley were the first to show this type of correspondence between the relative widths of the stripes and the durations of the magnetic reversals. The VMM hypothesis was confirmed within a few years when magnetic data were compiled from spreading ridges around the world. It was shown that the same general magnetic patterns were present straddling each ridge, although the widths of the anomalies varied according to the spreading rates characteristic of the different ridges. It was also shown that the patterns corresponded with the known timeline of Earth’s magnetic field reversals.
Concept Check: The Meaning of Magnetic Stripes
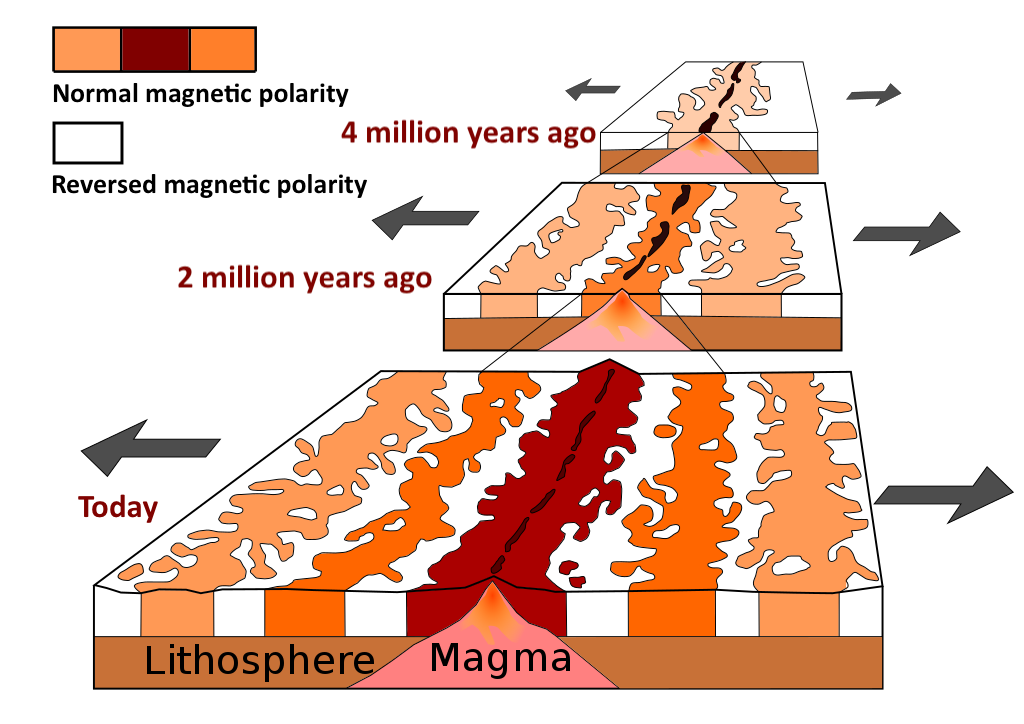
Fill-in-the-blank options:
- spreading centres
- north
- south
- normal
- crust
- reversed
- older
- symmetrical
To check your answers, navigate to the below link to view the interactive version of this activity.
Chains of Islands Progressively Aging Islands
In 1963, J. Tuzo Wilson of the University of Toronto proposed the idea of a mantle plume or hot spot — a place where hot mantle material rises in a stationary and semi-permanent plume, and affects the overlying crust. He based this hypothesis partly on the distribution of the Hawai’ian and Emperor Seamount island chains in the Pacific Ocean (Figure 4.18). The volcanic rock making up these islands becomes progressively younger toward the southeast, culminating in the island of Hawai’i itself, which consists of rock that is almost all younger than 1 Ma.
![Figure 4.15 The ages of the Hawaiian Islands and the Emperor Seamounts in relation to the location of the Hawaiian mantle plume [SE. Basemap from the National Geophysical Data Centre, accessed at: http://en.wikipedia.org/wiki/Hotspot_(geology)#/ media/File:Hawaii_hotspot.jpg.]](https://opentextbc.ca/physicalgeologyh5p/wp-content/uploads/sites/360/2021/08/image0312.png)
Wilson suggested that a stationary plume of hot upwelling mantle material is the source of the Hawaiian volcanism, and that the ocean crust of the Pacific Plate is moving toward the northwest over this hot spot. Near the Midway Islands, the chain makes a pronounced change in direction, from northwest-southeast for the Hawai’ian Islands, to nearly north-south for the Emperor Seamounts. This change has been ascribed to a change in direction of the Pacific Plate moving over the stationary mantle plume. An alternative hypothesis is that rather than the Pacific Plate having undergone a sudden change in motion, the plume itself has moved at least 2,000 km south over the period between 81 and 45 Ma (Tarduno et al., 2003).
There is evidence of many such mantle plumes around the world (Figure 4.19). Most are within ocean basins, including places like Hawai’i, Iceland, and the Galapagos Islands, but some are under continents. One example is the Yellowstone hot spot in the west-central United States, and another is the one responsible for the Anahim Volcanic Belt in central British Columbia. It is evident that mantle plumes are very long-lived phenomena, lasting for at least tens of millions of years, and possibly for hundreds of millions of years in some cases.
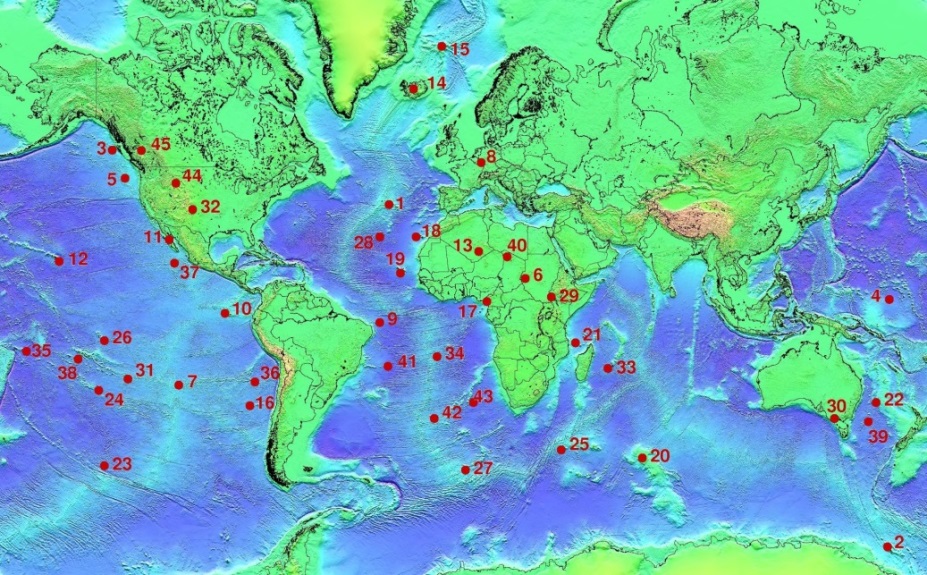
Transform Faults
Oceanic spreading ridges appear to be curved features on Earth’s surface, but the ridges are in fact composed of a series of straight-line segments, offset at intervals by faults perpendicular to the ridge (Figure 4.20). In a paper published in 1965, Tuzo Wilson termed these features transform faults.
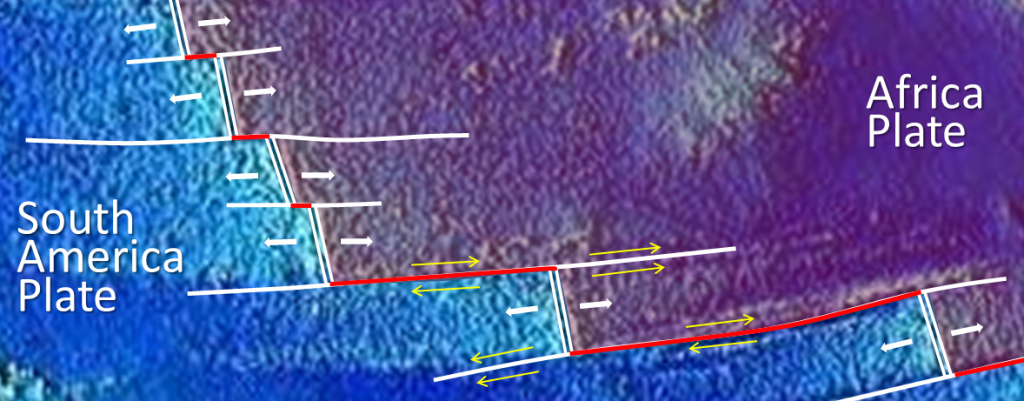
In the same 1965 paper, Wilson introduced the idea that the crust can be divided into a series of rigid plates, and is thus responsible for the term plate tectonics.
Paper Transform Fault Model
J. Tuzo Wilson used a paper model similar to the one in Figure 4.21 to explain transform faults to his colleagues. To use this model, print Figure 4.21, cut around the outside, and then slice along the line A-B (the fracture zone) with a sharp knife. Fold down the top half where shown, and then pinch together in the middle. Do the same with the bottom half. When you’re done, you should have two folds of paper extending downward as in Figure 4.22.
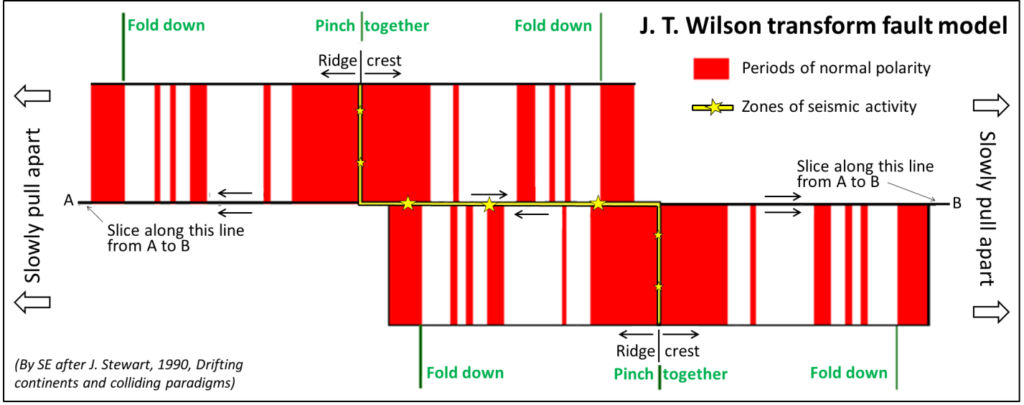
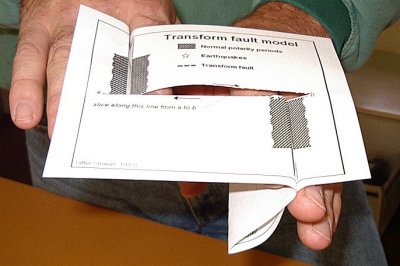
Find someone else to pinch those folds with two fingers just below each ridge crest, and then gently pull apart where shown. As you do, the oceanic crust will emerge from the middle, and you will see that the parts of the fracture zone between the ridge crests will be moving in opposite directions (this is the transform fault) while the parts of the fracture zone outside of the ridge crests will be moving in the same direction. You will also see that the oceanic crust is being magnetized as it forms at the ridge. The magnetic patterns represent the last 2.5 Ma of geological time.
There are other versions of this model available at Paper models of transform faults by Steven Earle. For more information see Earle (2004).
References
Earle, S. (2004). A simple paper model of a transform fault at a spreading ridge. Journal of Geoscience Education, 52, 391-392.
Raff, A., & Mason, R. (1961) Magnetic survey off the west coast of North America, 40˚ N to 52˚ N latitude. Geological Society of America Bulletin, 72, 267-270.
Stewart, J. A. (1990). Drifting continents and colliding paradigms. Indiana University Press.
Tarduno, J. A., Duncan, R. A., Scholl, D. W., Cottrell, R. D., Steinberger, B., Thordarson, T., Kerr, B. C., Neal, C. R., Frey, F. A., Torii, M., and Carvallo, C. (2003). The Emperor Seamounts: Southward Motion of the Hawaiian Hotspot Plume in Earth’s Mantle. Science, 301(5636), 1064–1069. DOI: 10.1126/science.1086442
Wilson, J. T. (1965). A new class of faults and their bearing on continental drift. Nature, 207, 343-347.
- Ted Irving later set up a paleomagnetic lab at the Geological Survey of Canada in Sidney BC, and did important work on understanding the geology of western North America. ↵